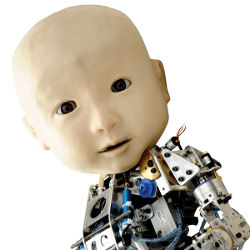
There are many different kinds of robots: factory automation systems that weld and assemble car engines; machines that place chocolates into boxes; medical devices that support surgeons in operations requiring high-precision manipulation; cars that drive automatically over long distances; vehicles for planetary exploration; mechanisms for powerline or oil platform inspection; toys and educational toolkits for schools and universities; service robots that deliver meals, clean floors, or mow lawns; and “companion robots” that are real partners for humans and share our daily lives. In a sense, all these robots are inspired by biological systems; it’s just a matter of degree. A driverless vehicle imitates animals moving autonomously in the world, a factory automation system is intended to replace humans in tasks that are dull, dirty, or dangerous. The term “robot” itself is anthropomorphic as it is derived from the Czech word “robota,” which is generally translated as “drudgery” or “hard work,” suggesting the analogy to people.
Key Insights
- Recent developments in the field of bio-inspired robotics have been centered on the idea that behavior is not only controlled by the brain, but is the result of the reciprocal dynamical coupling of brain (control}, body, and environment.
- Future generations of robots will be bio-inspired, have soft bodies composed of soft materials, soft actuators and sensors, and will be capable of soft movements and soft and safe interaction with humans.
- Progress in bio-inspired robotics can only occur when various technologies—computation, sensors, actuators, materials—are integrated and can be made to smoothly cooperate to achieve desired behaviors.
- Because part of the control in bio-inspired soft robotic systems is outsourced to morphological and material properties, novel design principles for “orchestrating” behavior must be developed.
- Bio-inspired soft robotics technologies might entail a quantum leap in the engineering of robots with complex skill sets capable of dexterous manipulation and safe cooperation with humans.
However, if we look inside these robots, we find that for the better part, they function very differently from biological creatures: they are built from metal and plastic, their “brains” are microprocessors, their “eyes” cameras, their “ears” microphones, and their “muscles” electrical motors that sit in the joints. Humans and other animals, by contrast, are built from biological cells; they have muscles made of fiber-like material that pull tendons anchored to the bones of the head, arms, fingers, and legs; they have a soft skin covering the entire body; their sense of sight relies on a retina that spatially encodes visual information and performs a lot of processing right at the periphery; and their brains are made up of an extremely intricate network of neurons.
Biological inspiration does not imply that we attempt to copy nature. Rather, the goal is to understand the principles underlying the behavior of animals and humans and transfer them to the development of robots. For example, when we walk, our muscles constantly change their stiffness: they are loose when the leg is swinging forward; they stiffen up when we put the foot on the ground. This idea can be employed on robots without having to apply the same “technology” as biological muscles. The important principle is the smooth change in stiffness achieved, for example, with tunable springs, that is, actuators where the spring constant can be dynamically varied.
The degree to which robots resemble biological systems can be used as one of the “dimensions” for characterizing the “robot space” in which we can position the different kinds of robots that exist (see Figure 1). The other dimension we use to characterize the robot space is behavioral diversity or complexity. Behavioral diversity designates the variety of actions a robot is capable of executing. Take a stuffed animal, a Teddy (Figure 1c), as an extreme case: It does not move, so its behavioral diversity is very low, zero in fact. The possible actions of a vacuum cleaning robot such as Roomba are also quite restricted: it can turn left or right, it can turn on and off its vacuuming device, and it can dock onto the charging station if the battery charge starts getting low. By contrast, animals and humans can do many different kinds of things: they can walk, run, look around, find their way to a food source and back to the nest or homes, handle objects such as food and materials for building a nest or house, they can hunt, eat, engage in courtship behavior, and they can reproduce. Note that behavioral diversity is different from technological (or biological) complexity: The autonomous car Stanley (Figure 1b), which won the 2005 DARPA Grand Challenge, has low behavioral diversity—it can, in essence, speed up, slow down, turn left and turn right—but with its sophisticated sensory systems and its advanced computational facilities, it has extremely high technological complexity.
Diversity is also expressed in the environments in which robots operate. In a factory, for example, the environment is well known so that the robots’ movements can, in principle, be preprogrammed down to the last detail. By contrast, service and companion robots have to function in the real everyday world, such as a city street, a shopping center, a soccer field, a school, or an amusement park where everything changes rapidly, with people rushing about so that individual movements can no longer be planned ahead of time. Humans can function easily and without effort in such real-world situations because they have evolved to cope with environments where predictability is very limited. Moreover, the real world is full of different kinds of objects such as plates, glasses, eggs, tomatoes, bags, hammers and nails, pets, children, and cars that require very diverse sets of skills to interact with and manipulate: Glasses are rigid but fragile and if they are filled with drinks, they must be handled with care so the liquid does not spill. Especially challenging is the treatment of eggs whose shells are very brittle and making a fried egg requires special abilities. The imitation of most of these capabilities has to date defied automation.
The kinds of next-generation robots we discuss in this article are those with a high behavioral diversity and a high degree of bio-inspirationa (see Figure 1a). Similar to humans, such robots will, in addition to hard components such as bones, have soft bodies made out of soft materials, soft actuators (muscles, tendons, and ligaments) and sensors (soft, deformable skin with touch and temperature receptors), and will be capable of soft movements and soft interaction with people. It is our contention that fast, efficient and robust behavior can be achieved by adequately exploiting material properties in particular “softness:” tails of fish and wings of birds passively adapt to the environment during locomotion, the elasticity of the muscle tendon system supports coping with impact and moving over rough ground, and the deformable, soft tissue in the hand and on fingertips enormously simplifies the task of grasping hard objects. In other words, soft materials may enable us to automate tasks that are beyond the capacities of current robotic technology.
However, introducing softness into the design of robots leads to design issues that differ completely from the classical ones known from “hard” type engineering, and we will thus need to elaborate a novel set of design principles that can act as a bridge between hard and soft type engineering. The main ideas are borrowed from biology and from the concept of embodiment used to characterize the role of the body in intelligent behavior. We therefore claim that with deep understanding of the far-reaching implications of embodiment, we will greatly accelerate the development of soft robotic systems.
In this article we briefly review a selection of recently developed bio-inspired robots and derive some of the enabling technologies and principles that have been, explicitly or implicitly, incorporated into their design. We then look at the evolution of the field and identify some of its great challenges. Finally, we argue that we might in fact be on the way to a new industrial revolution.
Biologically Inspired Robots
The biological world is immensely diverse—the total number of named species populating our planet is almost two million. Such variability of life forms provides an exceptional source of inspiration for scientists and engineers, which is reflected in the myriad of robots that have been developed. Bio-inspiration has been derived from fungus-like organisms such as the slime mold42 all the way to primates such as monkeys and human beings.18,21,31 Biology contains especially rich knowledge for robotics in disciplines such as neuroscience (in particular, computational neuroscience), biomechanics (the science of movement), and systems biology.
Building on the seminal work of Rodney Brooks5,6 at MIT in the 1980s, which was the starting point for the field of “embodied intelligence” in artificial intelligence and robotics, a striking variety of bio-inspired robots have been built over the last 20 years. Bio-inspiration has driven research and applications on robot locomotion (crawling, walking, running, climbing, jumping, swimming, and flying), navigation and orientation behaviors, spatial memory formation, exploration, environmental monitoring, manipulation, imitation, and cooperation, among others.
Many of these robots have been used to study and test models of natural neural information processing; for instance, to explore the switching between swimming and walking observed in salamanders20 or to investigate adaptive dynamic walking on irregular terrain30 (for recent surveys, refer to Floreano and Mattiussi,14 Meyer and Guillot,32 and Pfeifer et al.36). Much attention has also been devoted to emulating navigation and orientation behavior. Examples abound and include visual homing inspired by how bees or wasps find their way back to their nests, cricket phonotaxis (how female crickets move toward the mating sounds of males in highly rugged and noisy environments45), and spatial memory formation by modeling place fields and head-direction cells that account for the remarkable navigational skills of rodents.48 Soccer robots are a special breed of robots that are bio-inspired in a broad sense, where at least until recently, the capabilities for rapid movement, kicking skills, perception, cooperation, and strategyb are of greater interest than external shape and appearance.
A recent trend in bio-inspired robotics is to simplify the typically computationally intensive neurally inspired control through clever morphological design and use of functional materials.9,12,19 A case in point is aquatic locomotion. The key to the control of underwater robots, which mostly have a multi-segmented structure, is the translation of computational activity into torques propagating through the individual segments, so the resulting forces lead to forward movement. An alternative strategy to building such robots is to under-actuate them, that is, to drive only some of the joints, leaving the others passive, and “outsource” as much as possible to the morphology and bio-inspired materials. Simply moving a tail back and forth gives rise to surprisingly lifelike movements if the material of the tail fin is chosen appropriately; such materials allow the “tuning” of the mechanical properties of the fin in a way that optimally distributes the hydrodynamic forces over the fish’s body during propulsion and maneuvering13,50 (see Figure 2i).
As for swimming, also for flying one possible avenue might be the exploitation of passive dynamics and the morphology of bio-inspired materials8,44 (see Figure 2j). Take an insect wing during hovering flight. Its material properties in terms of resilience, stiffness, and deformability are essential to generate adequate lift in the absence of any forward velocity. It has been observed that the shape of the wing changes greatly when moving back and forth through the stroke plane. Although such change in shape could in principle be actively controlled, it is more efficient and faster if the intrinsic material characteristics are exploited and control is outsourced to the morphological and material properties of the wing. An additional advantage of this solution is that the wings can be made much lighter because less actuation is required.
Finally, materials can also be exploited for climbing, as beautifully showcased by the uncanny climbing skills of geckos that can dash up smooth walls and walk across ceilings with great ease. The geckos owe their sticky feet to the structural properties of their toes, which are covered with millions of nanoscale hair-like stalks branching into hundreds of tiny endings. The use of micropatterned fibrillar dry adhesives inspired by gecko foot morphology is bound to lead to impressive advances in the construction of robots that can climb vertical or inverted surfaces of all kinds.43
A field that has also received considerable attention over the past decade is humanoid robotics. Substantial efforts have been directed toward engineering robots with high behavioral diversity capable of performing a large variety of “human-oriented” tasks such as assisting the elderly (healthcare, physical support, shopping, leisure), doing household chores (washing the dishes, cooking dinner, and ironing), helping workers on assembly lines, as well as surveillance, and entertainment. Much progress has been made in the study of basic abilities such as bipedal locomotion;19 manipulation;12,24 understanding the surrounding environment, including the recognition of objects, people, and other robots;1 and social interaction3,33 (Figure 2g). Because such robots need to operate in environments built for humans, a reasonable morphology of choice is humanoid or anthropomorphic.
Humanoid robots often have highly sophisticated sensory-motor systems, and as a consequence they are confronted with the difficult problem of processing potentially large amounts of information in real time. Although much research has been conducted on learning in the real world, especially in the fields of artificial intelligence and cognitive robotics,41 the tasks and environments have, for the most part, been of relatively limited complexity. Potential reasons might be the size of the search spaces for learning optimal decision policies in realistic scenarios and the slow operating speed of real robots, which implies that the direct transfer of traditional algorithm-based machine-learning techniques to robots is not straightforward. A possible solution to the problem might be imitation learning, in which robots learn from humans or other robots.1,3,11 This idea has a special appeal because imitation is a powerful mechanism for reducing the search spaces associated with learning in the real world, which could lead to robots that will require only a minimal amount of programming. It is important to note that, in the context of learning by imitation, the embodiment has to be carefully considered because such learning would not work as efficiently if the mechanical properties of robot bodies were completely different from the ones of the teacher (humans).
Let us now draw some conclusions concerning technologies that have in fact enabled the construction of these robots, and about principles that have—explicitly or implicitly—been employed in their design and construction.
Enabling Technologies
As we move toward more highly complex anthropomorphic robots, a number of enabling technologies are increasingly important for progress to take place. In a complex robot such as ECCE (Figure 3) a wide range of technologies, which are complex in themselves, have to be integrated and they must smoothly cooperate to achieve the desired behaviors: sensors, actuators, materials for body limbs and skin, and computational resources. This is especially true for bio-inspired soft robotics, where the physical properties of the body, for instance the elasticity of the actuators and the deformability of the surface of the hands and the feet, are instrumental to cope with impact in walking and for safe interaction between man and machine. Moreover, because for the better part, bio-inspired robots are mobile, they require some kind of portable power source, and if they are to interact closely with humans in everyday life, they should not be too big. This implies on the one hand the need for efficient power sources and actuators, and on the other that the machines should be light and if possible also compact.
Let us start by reviewing recent developments in biologically inspired sensory systems. Due to space constraints, we focus on visual and tactile sensing and do not review other sensor modalities (for example, hearing, smell, taste, and pain).
The field of computer vision has a long history and has produced a large number of applications, for example in surveillance, quality control for electronic circuit boards, image search, automatic driving, traffic enforcement cameras, face recognition systems, among others.40 Biologically inspired vision attempts to replicate—and even go beyond—some of the amazing features of the human perceptual system, such as its extremely high reaction speed, its capacity for recognizing objects at varying distances, orientations, and partial occlusions, its ability for reliable interpretation of the environment, for localization, for providing a stable image while moving, and its adaptivity to dramatic changes in lighting conditions (from bright sunlight to near darkness). All these competences are still unmatched by today’s vision-based robots.
One potential avenue toward truly bio-inspired vision systems is neuromorphic engineering—a discipline that aims at realizing artificially engineered systems inspired by the physical foundations, function and structure of biological nervous systems. Novel (neuromorphic) silicon retinas offer advantages over conventional cameras, such as a higher range of sensitivity, speed, color detection, and the discounting of shadows—an ability that is currently under development27 (see Figure 4c). These silicon retinas mimic the computational principles of biological vision and rely on a continuous stream of asynchronous events from individual pixels—equivalent to the spikes delivered from the retina to the brain via the optic nerve—yielding activity that represents, for instance, scene contrasts and contrast changes. Such activity-driven, event-based systems have been tested in many applications where rapid responses are required such as in tracking systems, soccer playing robots, and pole balancing tasks.7 At this point in time, vision systems with artificial retinas are still laboratory prototypes but because of their many desirable characteristics, we expect the technology to expand rapidly in the near future. Similar neuromorphic technologies have also been successfully applied to silicon cochleae.27
One of the most important organs of humans (and many animals) is the skin, and there is a vast amount of evidence demonstrating its significance in survival and intelligent behavior. The skin is equipped with touch and temperature sensors (and pain receptors, which we will not discuss further in this article) that are distributed over the entire organism. Their distribution is not homogeneous but varies greatly depending on their position on the body: in the hand and on the fingertips, in the face and on the lips, their density is very high (about 250 mechanoreceptors per sq. cm in the fingertips alone), whereas on the back, for example, it is low (see Figure 4b). Skin is soft and deformable, but at the same time robust and waterproof. Because of the central importance of tactile sensing for learning, manipulation, and tool use, improvements in skin technology are likely to lead to a quantum leap in soft robotics. Various technologies have been suggested (for a detailed review of tactile sensing in robotics, see Dahiya et al.10). The approach to mimicking the pressure sensors in the skin on the fingertips shown in Figure 4b is based on force-sensitive resistors built into a flexible fabric. The fabric can be freely bent without obstructing the function of the sensors that is, of course, essential because the skin has to be placed everywhere on the hand (and perhaps other parts of the robot). In CB2, the child robot1,33 (see Figure 1g), the entire body is covered with skin, which is important because skin is a prerequisite for forming an image of one’s own body while growing up.17
Let us now switch to actuator technologies, keeping in mind that sensor mechanisms are often integrated with movement systems, for example, in the human muscle-tendon complex, which not only actuates the body but also incorporates sensing for force and length of muscles via muscle spindles and Golgi tendon organs. Proper actuation for bio-inspired robots has turned out to be a notorious issue and has so far been a decisively limiting factor.19 Biological muscles have many desirable properties: they have a high contraction ratio, they are energy efficient, they are intrinsically compliant, and their stiffness can be varied smoothly and dynamically. In order to deal with impact, the stiffness is increased, as pointed out earlier. Most of the robots shown in Figure 2 can, to some extent, change the compliance of their actuators, which they exploit to take over some of the control functions (coping with impact, smooth manipulation, and interaction). One type of actuator that dynamically changes its compliance is the pneumatic artificial muscle or fluidic air muscle (Figure 4a). This actuator is a contractile device that consists of an internal bladder surrounded by a braided mesh shell attached at either end to fittings. When the internal bladder is pressurized, the actuator shortens and its stiffness increases. In other words, pneumatic artificial muscles are intrinsically compliant, and their compliance can be controlled via the global parameter of air pressure. Another type of compliant drive system used, for example, in the ECCE-Robot (Figure 3) or in MIT’s Domo,21 is the series-elastic actuator, where an elastic element (for example, a spring) is placed between the output of a motor’s gear train and the load. Many actuation technologies have been proposed, but none has achieved the performance level of biological muscles. Note that using standard electrical motors in the joints the change in compliance can only be achieved at a high cost in terms of electronics and computation.
Because of the central importance of tactile sensing for learning, manipulation, and tool use, improvements in skin technology are likely to lead to a quantum leap in soft robotics.
Highly complex robots such as ECCE require elaborate middleware technology, that is, computer software that acts as a bridge between the multitude of heterogeneous and interconnected hardware and software modules composing them (sensors, actuators, software frameworks, programming languages, among others).c Robotics middleware is an active field of research and there are great hopes that it will allow robot designers to make the necessary abstractions to come to grips with the increasing complexity (and messiness) of robotic systems.
Perhaps more than any other factor, the lack of good power sources has limited the progress and the infiltration of mobile robots into our daily lives. Although computer processors and sensors have become cheaper and more powerful by the year, power generation and storage are still inefficient and, in the case of batteries, heavy, and slow to recharge, leaving engineers and scientists to dream of a day when they will have the juice to instill long lives into their creations. The BigDog robot (see Figure 2f) is driven by a combustion engine, which is convenient in terms of weight and fuel efficiency, but also very noisy and can only be used outdoors. The energy demands of legged robots are especially high compared to wheeled robots. But while wheels are effective in their energy use, they are limited to hard, smooth surfaces like roads. Recently, there have been some fascinating approaches to tackle the energy-efficiency issue in walking robots. One of the champions in this league is the somewhat odd looking Cornell Ranger that can go for 40.5 miles (65.2km) untethered on one battery charge alone in about 30 hours and 49 minutes.
Because most of the bio-inspired sensor and actuator technologies only exist in the form of laboratory prototypes and cannot be bought off the shelf, they often have to be manufactured individually for each robot. Rapid prototyping tools, such as 3D printers and freeform fabrication technologies represent a substantial enabling factor for this kind of engineering research. If, in addition, the 3D machines are capable of printing active, multi-material components, this will enable the automatic integration of sensors, logic gates, actuators, and power sources into complex structures, rendering the prototyping process even more effective.9,29,47
Due to the many unknowns, especially in the context of soft robotics, we also need powerful simulation tools so that ideas can be quickly tested before the systems are actually constructed in real hardware. In recent years, simulation methods that can be applied to robotics such as artificial evolution and physics-based modeling, have enormously improved (once again profiting from Moore’s Law) and are now standard development tools.4,14 This is not to say that the simulation of soft robots is an easy task; in fact, quite the opposite is the case and a lot of theoretical advances will be necessary before soft robots can be adequately simulated.
Progress in any of these technologies will lead to progress in bio-inspired robotics.
Design Principles
From the examples reviewed earlier, we can now derive a number of theoretical design principles that are beginning to make their way into the robotics community. These principles, which are compatible with current work in biomechanics, neuroscience, engineering, and embodied intelligence,34,35 can be seen as an extension of the work by Brooks mentioned earlier. We feel they represent an excellent starting point for future theoretical developments.
Physical embedding and task distribution. First, the behavior of a system is not merely the outcome of some internal control structure (such as the brain or a microprocessor), but it is also shaped by the environment in which the system is physically embedded, and by its morphological and material characteristics. For example, the elasticity of muscles can help to cope with the unevenness of the ground in walking, or the distribution of the receptor cells in the human eye already provides spatial information that substantially reduces processing cost in perception.25 Because of this “task distribution,” which is common to all humans and animals, a fundamental rethinking of our classical notion of control is required. There is a kind of trading space where computation is “outsourced,” so to speak, to the physical aspects of the agent, which implies that often the central control or computational effort can be reduced by orders of magnitude and the reaction times are dramatically shortened. In the jumping robot (Figure 2b), dealing with impact is delegated to the springy actuator system, in the Bionic Handling Assistant (Figure 2c), adaptation to the shape of the object is taken over by the mechanics of the gripper, which is based on the so-called fin-ray effect known from fish, and in the FILOSE robot (Figure 2i) finding the optimal shape for the tail fin is taken over by its material properties that, depending on the task, can be dynamically adjusted. In all these cases, very little central computational control is necessary.
Physical dynamics and information processing. Second, a direct link exists between embodiment and information: Coupled sensory-motor activity and body morphology induce statistical regularities in the sensory inputs, which simplifies the information processing task of the brain (or generally, the controller28,36). This information structure, which is the result of a physical interaction with the world, is at the core of how we can learn something about the environment. It depends strongly on the agent’s shape, the physical characteristics and the distribution of the different sensory systems, on the particular action and, of course, on the environment itself. If the robot shown in Figure 2a stimulates its whiskers by moving past an object, the sensory patterns induced will depend on the robot’s motion and on the object’s shape and surface characteristics. Or, when we walk, the environment travels across the visual field, a phenomenon called optic flow. It turns out that optic flow cannot only be easily calculated but is also extremely useful in navigation tasks. In order to avoid obstacles, optic flow is sufficient because nearby objects induce more optic flow than those further away. Note that the optic flow is induced through the agent’s own movement, it is not passively perceived. The walking robot, BigDog (Figure 2f) is continuously generating patterns of stimulation in the force sensors in its legs and its pressure receptors on its feet, which can be exploited for gait stabilization. The FILOSE fish robot (Figure 2i) creates stimulation in its flow sensors that delivers valuable information about how well its actions translate into forward movements.
Self-organization and emergence. Third, because robots are embodied, they can be viewed as complex dynamical systems that enable us to apply concepts of self-organization and emergence, rather than top-down control. Results from biology and bio-inspired robotics suggest that stable movement patterns, for example, can be productively characterized as attractor states. Agents display self-organization and emergence at multiple levels: induction of sensory stimulation, movement generation, exploitation of shape and material properties and interaction between individual modules and entire agents. For example, because both the swimming (Figure 2i) and the flying robot (Figure 2j) are under-actuated, that is, not all the degrees of freedom are driven, their bodies or wings will self-organize into the proper movements, namely, they will do the right thing even though they are not directly controlled. The movement of the Slimebot42 (see Figure 2h) is the result of a decentralized control in which each individual module is actuated independently from the others and whose global, emergent activity is coordinated through the physical interaction with the environment—yet another beautiful illustration of self-organization.
Complete agents. Finally, embodiment implies that all the components of the agent continuously interact and influence each other, and this needs to be kept in mind during the design process. For example, the movement capabilities must match those of the visual system: a snail with a powerful vision system such as the one of humans would not make any sense because, even if it had the brain to detect an approaching bird (which it has not), it could not do anything about it. The four legs of the BigDog robot are not only coordinated via internal connections, but because they are part of the same physical agent, each leg movement will instantaneously influence the values in the force and pressure sensors in the other legs. Also, induction of optic flow happens because the visual system is part of a complete agent that moves around in the world.
Although a certain consensus on these principles is emerging in the robotics and related communities, the new field of bio-inspired soft robotics is still lacking a firm foundation like control theory for traditional robotics and factory automation. The theory needs to be further developed, and we must work toward a better understanding of how behavior is “orchestrated” rather than controlled. This requires, among other issues, an elaboration of the trading space outlined here and implies a quantitative approach to morphology that, alas, is still missing.
In order to get a better idea of where the field of bio-inspired robotics might be going, let us briefly sketch its major challenges.
Robotics: Tough Challenges
To be sure, there has been enormous progress in robotics over the last 50 years—as can also be inferred from our review of some of the recent bio-inspired robots. In 1961, the first industrial robot, called Unimate, was commercialized and joined the assembly line of a General Motors plant; about 10 years later, there were already 3,000 robots operational worldwide; in 2009 the operational stock of industrial robots amounted to more than one million. If we look at mobile service robots, the progress is even more impressive. There were all of about 10 in the 1970s, mostly at universities and research institutes, whereas in 2009 almost 10 million had been sold worldwide, many of them used daily in homes for vacuum cleaning and lawn mowing, for entertainment and education, in hospitals, on disaster sites, or for surveillance purposes. According to the projections of the International Robotics Federation the sales for service robots for personal use are expected to increase sharply in the near future.
In spite of this quite impressive history, robot engineers have been jealously watching the rapid progress in computer technology and they have been asking why it is that microprocessor technology excels continuously—by now Moore’s Law has been in effect for several decades—whereas progress in robotics has been comparatively slow. The way most robots walk today is still very unnatural, their perceptual and manipulation skills are extremely limited, and they get easily confused if an unexpected situation occurs. We can only speculate about the reasons but this may help us to identify some of the major challenges.
First, robotics has become strongly interdisciplinary and involves many different fields of expertise, as well as researchers and engineers with a broad set of skills. Robots are highly complex systems. For instance, take humanoids such as ECCE, Domo, or Kojiro.38 They have a head, arms, hands, many joints, muscles (or motors), tendons, “eyes,” a skin with touch sensors, sensors to measure accelerations of individual body parts, force and length sensors for the tendons, and, of course, microprocessors with programs for perception, for controlling the robot’s movements, decisions, and learning behavior. In order for such robots to behave flexibly and efficiently, advances in sensors, actuators, energy, and propulsion technologies will be required. Moreover, and this represents a big theoretical challenge, the interaction among these components will have to be coordinated and orchestrated to achieve the desired behaviors. One of the reasons (biological) humans can walk and move so stably is that they are equipped with an enormous number of smoothly integrated sensors and a highly redundant muscle-tendon system. The walking robot BigDog has over 50 different sensors,37 but a humanoid, in order to competently walk, run, and manipulate objects, will need many more.
Given the enormous challenges we are up against when engineering robots for the real world, why should we do it in the first place?
There is an additional challenge, especially for mobile machines, that we alluded to earlier: Because robots are physical systems, they require energy to move, which implies there is a hard limit to how much their energy consumption can be reduced. Likewise, in the commercial airline industry, progress has also been slow, at least much slower than in microprocessor technology, not only because many technologies have to come together to make it work, but because there is a minimum amount of energy required to transport humans and the airplane itself, both physical systems. Power consumption for microprocessors is also an issue but so far the theoretical limits in terms of energy required for processing and storing information have not been reached. In robotics, energy consumption and storage is a notorious problem.
So far, we have been stressing the fact that focusing on computational aspects alone will not be sufficient if we are to build soft, real-world machines. However, computation has formed, and will form in the future, a core component of any intelligent machine: perception, decision making, problem solving, and action generation will always require sophisticated kinds of computation. What the new theoretical insights from the area of embodied intelligence are bringing into the discussion is, as introduced earlier, the idea that certain aspects of the computation can be off-loaded to morphological and material components. This not only simplifies computation but also increases reaction speed—physical processes are often much faster than computational ones. Given the experience accumulated within computer science over a half century, it seems natural to join forces in an attempt to devise a novel concept of computation—which has also been called “morphological computation”—and see how the existing concepts could be transferred or extended to capture the additional phenomena in soft robotics, or to what extent new ideas are required. Undoubtedly, researchers will strongly benefit from this rich experience. We are convinced that computational thinking, as outlined in Jeannette Wing’s manifesto,46 will also apply to this challenge: it is not primarily about algorithms or programs, but a way of thinking about the world.
A New Industrial Revolution?
Given the enormous challenges we are up against when engineering robots for the real world, why should we do it in the first place? There are a number of key driving forces behind all these developments: scientific, social, demographic, and economic.
First off, scientists are continuously striving to overcome the limitations of current, “hard” robotics in order to move to the next level of behavior diversity. As argued throughout this article, this implies using a bio-inspired soft robotics approach. The ultimate scientific goal (and dream) is, of course, to have a robot with the skills of a human. Throughout the history of mankind, many attempts have been made to imitate humans and their abilities by exploiting the technologies and scientific insights at the time. From a scientific perspective, bio-inspired robotics is of great interest because with the soft technologies the area has become extremely interdisciplinary—it might in fact be one of the most interdisciplinary scientific fields around. The increased level of complexity of the subject matters requires novel ways of cooperation, supported by state-of-the-art collaboration technologies.
At the social level, mobile communication and computing technologies are rapidly spreading and increasingly penetrating our daily lives. This is now also beginning to happen with robot technology, for example, pet robots, service robots, health monitoring and support technologies for the elderly, toy robots, educational robots, and finally, it will be household and companion robots. As Bill Gates forcefully requested in his famous Scientific American article,16 “a robot in every home;” just as he demanded a computer in every home more than 20 years ago! Thanks to the ubiquitous use of mobile devices, technology in general is becoming more and more accepted as a close interaction partner. It is likely that the next step will be the integration of autonomous systems into our daily lives—a development that is already under way in Japan and Korea. In order to be able to perform tasks similar to those humans do, for example, shopping, cooking, cleaning the dishes, tidying up the children’s rooms, or pouring a glass of beer, they will have to be of the “soft” type.
Demographically, one of the core drivers is a rapidly aging population: Technologies must be developed to enable individuals to live autonomously for as long as possible. This includes service robots of all types, assistive technologies to compensate for lost bodily functions, “helpers” in everyday tasks, and monitoring systems for physiological conditions. Again, because Japan was the first to be strongly confronted with this issue, it is the country where the pertinent technologies are most advanced. Because similar tendencies are on their way in Europe and the U.S., massive R&D efforts in this direction backed by large investment commitments have taken off in these two regions.
This demographic development suggests two scenarios. The first and most commonly outlined is the companion robot—a robot capable of performing most or all of the tasks that humans can, as well as hold intelligent and personal conversations, and give medical, financial, and entertainment advice, and so on. Obviously, it must be strongly biologically inspired and must have extremely high behavioral diversity. Whether we will ever have such robots populating our homes is an open question, especially if we think of all the challenges we have pointed out here. Typically, such super-complex systems tend to be extremely expensive and fragile in their operation. Ultimately, it will be the markets that will decide on the viability of this concept. But there is an even more important caveat. Let us look at real biological humans for a moment. We humans can do many things, but for most sensory-motor tasks, there are—or will be—machines that perform the task faster, cheaper, and more precisely. For example, driving a screw the way we do is probably the most inefficient and slowest possible way: it would be much better to have a screwdriver motor that can rotate continuously. So why develop a machine, a companion robot that can perform many different things but nothing really well?
We humans can do many things, but for most sensory-motor tasks, there are—or will be—machines that perform the task faster, cheaper, and more precisely.
This implies an alternative scenario, one in which we will have many specialized machines specifically geared toward particular tasks such as vacuum cleaners, lawn mowers, dish-handling machines, shopping assistants on wheels, automated waiters, among others. While these robots will for the better part not be humanoid, they will, depending on the particular tasks, employ soft robotics principles and technologies. In other words, what we have learned from building super-complex humanoids can be exploited and transferred to specialized machines. Festo’s bionic handling assistant or the BioRob arm,24 designed for manipulation and safe interaction with humans are not humanoid but incorporate soft robotics concepts. But, as suggested earlier, the jury is still out on which scenario will in fact materialize.
The last driving force, and a very strong one, is economic. Recently, there has been a lot of talk in the media that in certain areas in China a shortage of qualified labor is beginning to emerge and workers are asking for higher salaries and better working conditions, and they even risk going on strike. The country’s one-child policy, for the better or the worse, is beginning to show its effects. Thus, it can no longer be the default strategy for European and U.S. companies to outsource manufacturing and assembly tasks that are beyond the current level of factory automation to low labor cost countries. Foxconn, for example, produces and packages iPhones and iPads for Apple and components for many other electronic equipment manufacturers. Western enterprises are starting to ponder the idea of re-insourcing some of the production back into their own countries—in particular, by empowering small- and medium-sized companies with next-level low cost but highly refined automation solutions. However, in order to remain competitive, the initial capital requirements must be reduced and the degree of automation increased, such that tasks currently beyond what existing manufacturing methods can achieve in terms of price and speed, can be automated as well. Moreover, there is the additional requirement of flexibility and versatility: The tasks must be switchable quickly without the need for a lot of tedious reprogramming.
In spite of enormous advances in robotic tools to support task switching to date, the rapid adaptability of humans to new tasks remains unparalleled. And this is where the soft approach to robotics might in fact come into play, because it is likely to lead to robots with complex skill sets capable of dexterous manipulation and safe interaction and cooperation with humans, for example, where humans could teach tasks to robots by demonstration. Rethink Robotics and Adept located in the U.S. and pi4_robotics from Germany are companies specializing in next-level factory automation. So, what we have learned and are still learning by building full-fledged, super-humanoid robots might in fact have spinoffs that could ultimately lead to a new industrial revolution.
Figures
Figure 2. Recent bio-inspired robots.
Figure 3. The anthropomimetic humanoid robot ECCE (Embodied Cognition in a Compliantly Engineered Robot).
Figure 4. Bio-inspiration and enabling technologies.
Figure 5. An illustration of the design principles for bio-inspired robots.
Figure. Affetto, the robot baby created by researchers in the Asada Laboratory at Osaka University, is being used to study cognitive robotics. For more on Affetto, visit http://projectaffetto.blogspot.jp/p/project.html
Join the Discussion (0)
Become a Member or Sign In to Post a Comment