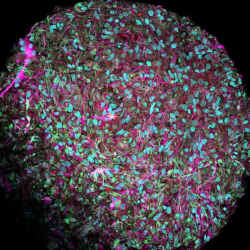
Biocomputing sits at the intersection of computer science, biology, and engineering. Researchers in the field seek to exploit the inherent molecular and chemical qualities of biological materials—including microorganisms like bacteria and fungi and cell components like DNA—to advance computing. Demonstrated and potential applications include performing computational tasks, data storage and retrieval, and the construction of novel hardware.
Proponents argue that biocomputing has advantages over conventional electronic methods. For example, the technology does not rely on fast-heating silicon microchips, making it more energy efficient, and many living materials have the useful ability to self-repair.
The origins of biocomputing date back to the 1990s, when Turing Award recipient and computer scientist Leonard Adleman demonstrated that DNA molecules could be encoded to carry out computational tasks. Recent developments in synthetic biology and nanobiotechnology have advanced the field by permitting the manipulation of biological materials on a nanoscale. Breakthroughs are happening globally and range from bacteria-driven functionalities to ongoing work on potentially disruptive technologies based on fungal mycelium—and human brain cells.
Bacterial devices
Bacteria are microorganisms that display behaviors—like gene expression and quorum sensing, a type of chemical communication—that can be genetically engineered to perform computing tasks.
Sangram Bagh and Rajkamal Srivastav, biophysicists at the Saha Institute of Nuclear Physics in Kolkata, India, have developed a logically reversible double Feynman gate using molecular engineered bacteria in an Artificial Neural Network (ANN) architecture. Their 3-input-3-output double Feynman logic gate using laboratory engineered, non-pathological E. coli cells is, say the researchers, the first realization of a double Feynman gate using living cells.
Bagh and Srivastav developed "cellular devices" by creating synthetic genetic networks inside E. coli and constructing a single-layer artificial network-type architecture with the engineered bacteria, which they describe as 'bactoneurons'. The double Feynman gates were generated when the bactoneurons were arranged in this architecture. The cellular devices' input signals were produced using extracellular chemicals, Bagh explains; "This input signal is like a zero or one. It is present or not, and then you get an output." The output, in this case, is the expression of three fluorescent proteins.
Other bacteria-driven biocomputing solutions are emerging: a team from the Walton Institute and the Tyndall National Institute in Ireland, and the University of Essex in the U.K., have proposed Bacterial Molecular Computing on a Chip (BMCoC) using microfluidic and electrochemical sensing technologies. Researchers at the Centro Nacional de Biotecnología (CSIC) and Complutense University of Madrid (UCM) in Spain and the Diego Portales University in Santiago, Chile, have shown "the possibility" of programming synthetic bacteria using a perceptron neural network. In Boston University´s Oliveira Lab, researchers are developing programmable microbial communities embedded in microfluidic devices.
Bacteria are single-celled and microscopic, but some biocomputing experiments are based on more complex living structures.
Fungal substrates
Researchers at The Unconventional Computing Laboratory (UCL) of the University of the West of England in the U.K., Utrecht University in the Netherlands, the Centre for Information Technology and Architecture (CITA) at The Royal Danish Academy in Denmark, and Italian mycelium-based technologies organization MOGU are partnering in an ongoing EU-funded project entitled Fungal Architectures, which aims to build a "fully integrated structural and computational living substrate using fungal mycelium for the purpose of growing architecture."
Adam Adamatzky, the director and founder of the UCL, listed the advantageous qualities fungi offer for his research:
- "Fault tolerance and self-regeneration: it is impossible to destroy all mycelium underground.
- Reconfigurability: we can program growth of mycelium networks using repellents and attractants.
- Evolvability— as all creatures do, fungi evolve.
- Very low energy consumption—fungi are computers which live on rotten trees."
Last year, Adamatzky and colleague Nic Roberts, also of the UCL, demonstrated mining logical circuits in fungi, and in 2021, he published a paper with colleagues at CITA introducing "fungal electronics": living electronic devices, including chemical and photosensors and oscillators, made of pure mycelium or mycelium-bound composites. Says Adamatzky, "I have life-long interest in implementing computing devices from unusual substrates."
An equally unusual but promising biocomputing material is slime mold, Physarum polycephalum. Once classified as fungi, it is now understood to be a distinct biological entity. At ACM´s Symposium on User Interface Software and Technology (UIST) last year, Jasmine Lu and Pedro Lopes of the University of Chicago's Human Computer Integration Lab presented a slime mold-integrated smartwatch. In other developments, an international team from Wenzhou University, China, Duy Tan University, Vietnam, the University of Tehran, Iran, and Torrens University Australia have proposed a Slime Mold algorithm (SMA) for tackling optimization problems.
A new frontier?
The newest area of biocomputing is perhaps also the most surprising. The Organoid Intelligence (OI) project brings together researchers from Johns Hopkins University, the Howard Hughes Medical Institute, and the University of California San Diego in the U.S., Australia's Cortical Labs and the universities of Luxembourg and Konstanz in Germany. The aim of the project is to use three-dimensional (3D) cultures of brain cells, called brain organoids, to build powerful biocomputers.
In February, the team released a roadmap for establishing OI as a new scientific discipline. Published in Frontiers in Science, the paper defines the current state of OI, as well as some of the scientific, technological, and ethical challenges it faces.
OI-based biocomputing seeks to deploy human stem-cell-derived brain organoids that can memorize and compute input. This, say the researchers, will support supercomputing through advances in processing power, data efficiency, and storage capabilities.
Thomas Hartung, a toxicologist at Johns Hopkins University, says 3D brain organoids can already be produced. They possess attributes that can be exploited in OI-based biocomputing, he said, such as high cell density, spontaneous electrophysiological activity/ reactivity, and enhanced levels of glial ("helper") cells that support neurons in biological learning tasks.
"You need the helper cells, the glial cells, to establish long-term memory. They have to take away all of the connections which are not useful; pruning of synapses we call this," Hartung explains.
However, current brain organoids consist of less than 100,000 cells. "They're tiny, tiny, tiny," says Hartung. To compute and memorize usefully, organoids need to be much larger. This presents a major practical challenge, as Hartung explains, "These models are rotting in the core if you make them larger."
Organoid scalability— up to around 10 million neural cells— is now a key research aim; so, too, are viability and durability. Work is taking place on multiple fronts, including the development of microfluidic perfusion systems to support homeostasis in the organoids, and addressing storage and processing challenges.
However, Hartung points out that proof of concept was established in October last year by Brett Kagan of the Cortical Lab in Melbourne, Australia. Kagan presented a synthetic biological intelligence (SBI) system called BrainDish that was created from living neurons based on embryonic mice and human-induced pluripotent stem cells (hiPSCs). The synthetic cell culture showed the ability to learn, and improve, gameplay in simulations of the 1970s arcade game Pong.
It may take some time, but many kinds of living things— from bacteria, slime mold, and mycelium to human brain cells—could one day power our devices.
K.J. Bannan is a writer and editor based in Massapequa, NY. She began her career on the PM Magazine First Looks team reviewing all the latest and greatest technologies. Today, she is a freelancer who covers business, technology, health, personal finance, and lifestyle topics.
Join the Discussion (0)
Become a Member or Sign In to Post a Comment